define chemistry?
Chemistry is a branch of physical science, is the study of the composition, structure, properties and change of matter.
What Is Chemistry?
Chemistry is the study of matter and energy and the interactions between them. This is also the definition for physics, by the way. Chemistry and physics are specializations of physical science. Chemistry tends to focus on the properties of substances and the interactions between different types of matter, particularly reactions that involve electrons. Physics tends to focus more on the nuclear part of the atom, as well as the subatomic realm. Really, they are two sides of the same coin.
Chemistry as science
Under the influence of the new empirical methods propounded by Sir Francis Bacon and others, a group of chemists at Oxford, Robert Boyle,Robert Hooke and John Mayow began to reshape the old alchemical traditions into a scientific discipline. Boyle in particular is regarded as the founding father of chemistry due to his most important work, the classic chemistry text The Sceptical Chymist where the differentiation is made between the claims of alchemy and the empirical scientific discoveries of the new chemistry
Prior to his work, though, many important discoveries had been made, specifically relating to the nature of 'air' which was discovered to be composed of many different gases. The Scottish chemist Joseph Black (the first experimental chemist) and the Dutchman J. B. van Helmontdiscovered carbon dioxide, or what Black called 'fixed air' in 1754; Henry Cavendish discovered hydrogen and elucidated its properties andJoseph Priestley and, independently, Carl Wilhelm Scheele isolated pure oxygen.
English scientist John Dalton proposed the modern theory of atoms; that all substances are composed of indivisible 'atoms' of matter and that different atoms have varying atomic weights.
The development of the electrochemical theory of chemical combinations occurred in the early 19th century as the result of the work of two scientists in particular, J. J. Berzelius and Humphry Davy, made possible by the prior invention of the voltaic pile by Alessandro Volta. Davy discovered nine new elements including the alkali metals by extracting them from their oxides with electric current.
British William Prout first proposed ordering all the elements by their atomic weight as all atoms had a weight that was an exact multiple of the atomic weight of hydrogen. J. A. R. Newlands devised an early table of elements, which was then developed into the modern periodic table of elements by the German Julius Lothar Meyer and the Russian Dmitri Mendeleev in the 1860s. The inert gases, later called the noble gases were discovered by William Ramsay in collaboration with Lord Rayleigh at the end of the century, thereby filling in the basic structure of the table.
chemical structure:
At the turn of the twentieth century the theoretical underpinnings of chemistry were finally understood due to a series of remarkable discoveries that succeeded in probing and discovering the very nature of the internal structure of atoms. In 1897, J. J. Thomson of Cambridge Universitydiscovered the electron and soon after the French scientist Becquerel as well as the couple Pierre and Marie Curie investigated the phenomenon of radioactivity. In a series of pioneering scattering experiments Ernest Rutherford at the University of Manchester discovered the internal structure of the atom and the existence of the proton, classified and explained the different types of radioactivity and successfully transmuted the first element by bombarding nitrogen with alpha particles.
His work on atomic structure was improved on by his students, the Danish physicist Niels Bohr and Henry Moseley. The electronic theory ofchemical bonds and molecular orbitals was developed by the American scientists Linus Pauling and Gilbert N. Lewis.
The year 2011 was declared by the United Nations as the International Year of Chemistry. It was an initiative of the International Union of Pure and Applied Chemistry, and of the United Nations Educational, Scientific, and Cultural Organization and involves chemical societies, academics, and institutions worldwide and relied on individual initiatives to organize local and regional activities.
Element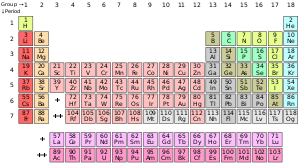
Standard form of the periodic table of chemical elements. The colors represent different categories of elements
A chemical element is a pure substance which is composed of a single type of atom, characterized by its particular number of protons in the nuclei of its atoms, known as the atomic number and represented by the symbol Z. The mass number is the sum of the number of protons and neutrons in a nucleus. Although all the nuclei of all atoms belonging to one element will have the same atomic number, they may not necessarily have the same mass number; atoms of an element which have different mass numbers are known as isotopes. For example, all atoms with 6 protons in their nuclei are atoms of the chemical element carbon, but atoms of carbon may have mass numbers of 12 or 13
Atom
A diagram of an atom based on the Rutherford modelThe atom is the basic unit of chemistry. It consists of a dense core called the atomic nucleus surrounded by a space called the electron cloud. The nucleus is made up of positively charged protons and uncharged neutrons (together called nucleons), while the electron cloud consists of negatively-charged electrons which orbit the nucleus. In a neutral atom, the negatively-charged electrons balance out the positive charge of the protons. The nucleus is dense; the mass of a nucleon is 1,836 times that of an electron, yet the radius of an atom is about 10,000 times that of its nucleus.
The atom is also the smallest entity that can be envisaged to retain the chemical properties of the element, such as electronegativity, ionization potential, preferred oxidation state(s), coordination number, and preferred types of bonds to form (e.g., metallic, ionic, covalent)..
Compound
Carbon dioxide(CO2), an example of a chemical compound
Main article: Chemical compoundA compound is a pure chemical substance composed of more than one element. The properties of a compound bear little similarity to those of its elements.[44] The standard nomenclature of compounds is set by the International Union of Pure and Applied Chemistry (IUPAC). Organic compoundsare named according to the organic nomenclature system.[45] Inorganic compounds are named according to the inorganic nomenclature system.[46] In addition the Chemical Abstracts Service has devised a method to index chemical substances. In this scheme each chemical substance is identifiable by a number known as its CAS registry number.
Molecule
A ball-and-stick representation of the caffeine molecule (C8H10N4O2).
A molecule is the smallest indivisible portion of a pure chemical substance that has its unique set of chemical properties, that is, its potential to undergo a certain set of chemical reactions with other substances. However, this definition only works well for substances that are composed of molecules, which is not true of many substances (see below). Molecules are typically a set of atoms bound together by covalent bonds, such that the structure is electrically neutral and all valence electrons are paired with other electrons either in bonds or in lone pairs.
Thus, molecules exist as electrically neutral units, unlike ions. When this rule is broken, giving the "molecule" a charge, the result is sometimes named a molecular ion or a polyatomic ion. However, the discrete and separate nature of the molecular concept usually requires that molecular ions be present only in well-separated form, such as a directed beam in a vacuum in a mass spectrometer. Charged polyatomic collections residing in solids (for example, common sulfate or nitrate ions) are generally not considered "molecules" in chemistry.
Main Branches of Chemistry
Although many would say that there are FIVE main branches of chemistry, namely: Physical, Analytical, Biochemistry, Organic and Inorganic chemistry many would argue that the science of chemistry actually links out to other branches or sub-branches that include Materials Chemistry, Theoretical Chemistry, Macromolecular (Polymer) Chemistry, Nuclear Chemistry, Metallurgy, Forensic Chemistry, Medicinal Chemistry and more.
It is important to note that often sub-branches fall under one or more of the main branches of chemistry.
Let’s start by taking a look at the 5 main branches of chemistry and then delve deeper into chemistry’s many sub-branches:
Analytical chemistry is the study involving how we analyze the chemical components of samples. How much caffeine is really in a cup of coffee? Are there drugs found in athlete’s urine samples? What is the pH level of my swimming pool? Examples of areas using analytical chemistry include forensic science, environmental science, and drug testing.
Analytical chemistry is divided into two main branches: qualitative and quantitative analysis.
Qualitative analysis employs methods/measurements to help determine the components of substances. Quantitative analysis on the other hand, helps to identify how much of each component is present in a substance.
Both types of analysis can be used to provide important information about an unidentified sample and help to identify what the sample is.
Biochemistry
The study of life or more aptly put, of chemical processes in living organisms. Biochemists research includes cancer and stem cell biology, infectious disease as well as membrane and structural biology and spans molecular biology, genetics, mechanistic biochemistry, genomics, evolution and systems biology.
Biochemistry, according to many scientists can also be explained as a discipline in which biological phenomena are examined in chemical terms. Examples are digestion and cellular respiration.
For this reason biochemistry is also known as Chemical Biology or Biological Chemistry.
Under the main umbrella of biochemistry many new sub-branches have emerged that modern chemists may specialize in solely. Some of these disciplines include:
- Enzymology (study of enzymes)
- Endocrinology (study of hormones)
- Clinical Biochemistry (study of diseases)
- Molecular Biochemistry (Study of Biomolecules and their functions).
There are also others like Pharmacological Biochemistry, Agricultural Biochemistry and more.
Click the informative links below to learn more about biochemistry:
Chemists in this field focus on elements and compounds other than carbon or hydrocarbons. Simply put, inorganic chemistry covers all materials that are not organic and are termed as non-living substances – those compounds that do not contain a carbon hydrogen (C-H) bond.
Compounds studied by inorganic chemists include crystal structures, minerals, metals, catalysts, and most elements on the periodic table. An example is the strength of a power beam used to carry a specific weight or investigating how gold is formed in the earth.
Branches of inorganic chemistry include:
- Bioinorganic chemistry (study of role of metals in biology)
- Coordination chemistry (study of coordination compounds and interactions of ligands)
- Geochemistry (study of the earth’s chemical composition, rocks, minerals & atmosphere)
- Inorganic technology (synthesizing new inorganic compounds)
- Nuclear chemistry (study of radioactive substances)
- Organometallic chemistry (study of chemicals that contain bonds between a metal and carbon – overlaps into organic chemistry)
- Solid-state chemistry/materials chemistry (study of the forming, structure, and characteristics of solid phase materials)
- Synthetic inorganic chemistry (study of synthesizing chemicals)
- Industrial inorganic chemistry (study of materials used in manufacturing. E.g.: fertilizers)
Organic chemistry
The study of carbon compounds such as fuels, plastics, food additives, and drugs. An opposite of inorganic chemistry that focuses on non-living matter and non-carbon based substances, organic chemistry deals with the study of carbon and the chemicals in living organisms. An example is the process of photosynthesis in a leaf because there is a change in the chemical composition of the living plant.
Organic chemists are often the ones who devise experimental methods to isolate or synthesize new materials, or to study their properties, and usually work and research in a lab. Some examples on the work they do include formulating a conditioner that keeps hair softer, developing a better drug for headaches and creating a non-toxic home cleaning product.
The branches of organic chemistry involve many different disciplines including the study of ketones, aldehydes, hydrocarbons (alkenes, alkanes, alkynes) and alcohols.
- Stereochemistry (study of the 3-dimensional structure of molecules)
- Medicinal chemistry (deals with designing, developing and synthesizing pharmaceutical drugs)
- Organometallic chemistry (study of chemicals that contain bonds between a carbon and a metal)
- Physical organic chemistry (study of structure and reactivity in organic molecules)
- Polymer chemistry (study of the composition and creation of polymer molecules)
The study of the physical properties of molecules, and their relation to the ways in which molecules and atoms are put together. Physical chemistry deals with the principles and methodologies of both chemistry and physics and is the study of how chemical structure impacts physical properties of a substance. An example is baking brownies, as you’re mixing materials and using heat and energy to get the final product.
Physical chemists would typically study the rate of a chemical reaction, the interaction of molecules with radiation, and the calculation of structures and properties.
Sub-branches of physical chemistry include:
- Electrochemistry (study of the interaction of atoms, molecules, ions and electric current)
- Photochemistry (study of the chemical effects of light; photochemical reactions)
- Surface chemistry (study of chemical reactions at interfaces)
- Chemical Kinetics (study of rates of chemical reactions)
- Thermodynamics/Thermochemistry (study of how heat relates to chemical change)
Quantum Mechanics/Quantum Chemistry (study of quantum mechanics and how it relates to chemical phenomena.
The periodic table of elements is a chart that outlines all the basic elements of chemistry that make up our world according to their atomic numbers, the number of electrons each element has, and their predominant chemical properties.
Each element is lined up from low to high atomic number, which simply refers to the number of protons it has. Most periodic table of elements charts are laid out in this fashion: A tabular grid of 18 by 7 that houses all of the major elements over another two rows of elements below it.
The table can also be broken down into 4 distinct parts or blocks: the s-block on the left, the p-block on the right, the d-block towards the middle and the f-block at the bottom.
The table rows are referred to as "periods" and the columns (s, p and d blacks) are called "groups." Some groups also have specific names such as the noble gases, or the halogens.
The name "periodic" table suggests that the table itself is open to being updated on a periodic basis, so it's not only used to uncover how each of the elements relate to one another but also to discover the characteristics of new elements or yet to be found or synthesized elements.
Therefore, the periodic table proves to be an important guide and resource when it comes to showcasing all the basic elements and studying chemical tendencies, and is commonly used not only in the science of chemistry but other fields of science as well.
Although other forms of the periodic table have been known to exist, Dmitri Mendeleev is typically recognized as the pioneer for publishing the first periodic table of elements in 1869. He designed the table to show similarities in the properties of the elements that were known back in the day. He also forecasted the properties of undiscovered elements back then and marked their place on the table, and in fact most of his claims and estimations proved true when the elements were discovered as time passed. Since the 1800's the periodic table has grown and improved with new elements being found and new theories explaining the way chemicals behave.
Elements from atomic number 1 to 118, hydrogen to ununoctium, have either been discovered or created. From all of these, all the elements you see up to californium occur naturally. Others have been created in labs. Chemists continue their pursuit to synthesize new elements way beyond ununoctium, but the presence of these synthesized chemicals having their place on the periodic table is still a question of continued disagreement and debate. Synthetic versions of elements that naturally occur in the earth have also been produced in chemical laboratories.


The above image of an eighteen column period table of elements structure is now the most commonly and broadly used format because it’s been so popular and widely accepted.
Also called the “long form” periodic table layout, it differs from Mendeleev’s short form design by removing the groups three to twelve and inserting them instead into the other major groups. The long form layout actually includes the actinides and the lanthanides in its structure instead of placing them below and away from the main table body. This wider layout table also adds two more periods, periods 8 and 9, and also incorporates the superactinides.

One thing to keep in mind is that the periodic table only documents chemical elements. It does not account for subatomic particles, or elements combined together such as mixtures and compounds. Each element's atomic number depends on how many protons it has in its nucleus. Isotopes are two or more variants of the same chemical element. They contain the same number of protons but carry a different number of neutrons in their nucleus. As the number of protons stays equal, the atomic number does not change. For example, carbon has three isotopes that occur naturally. Most have 6 protons but the number of neutrons can vary between 6 and 8. However, isotopes are always shown together under the element they belong to in the periodic table. Elements with no stable isotopes carry the atomic mass of the most stable isotope of that element, where the mass is displayed in parentheses.
Let’s take a closer look at the arrangement of elements in the table. As mentioned before, all the elements are arranged according to their rising atomic number, or their increasing number of protons. A new period or row begins when an electron shell gets its first electron. Groups or columns are arranged according to the number of electrons the atom carries. Also, elements that display comparable chemical characteristics usually also fall within the same column, and in the f and d blocks elements that lie in the same row to some extent also display similar characteristics. Therefore if you know the properties of a particular element it is fairly simple to figure out the properties of other elements that surround it in the table.
Here are some more facts about the periodic table:
According to the most updated version in 2012, the periodic table is said to have 118 elements. Of these 114 are official and have been named and documented by the International Union of Pure and Applied Chemistry (IUPAC).
Ninety-eight elements are naturally occurring out of which eighty-four are known as primordial elements. The remaining fourteen occur in decay chains of these primordial elements.
Even though elements like livermorium, flerovium and those listed from einsteinium to copernicium do not naturally occur in the earth and have been synthesized, they are still recognized by the IUPAC.
Other elements like 113, 115, 117 and 118 are known to be supposedly formulated in labs but the information has not yet been validated. Therefore these elements are only recognized by their element name, depending on their atomic number. As of the year 2012, there have been no reports of any element being synthesized keeping the count as of today at 118.
A SIMPLE VIEW OF ATOMIC STRUCTURE This page revises the simple ideas about atomic structure that you will have come across in an introductory chemistry course (for example, GCSE). You need to be confident about this before you go on to the more difficult ideas about the atom which under-pin A'level chemistry. The sub-atomic particlesProtons, neutrons and electrons.
| |||||||||||||||||
Beyond A'level: Protons and neutrons don't in fact have exactly the same mass - neither of them has a mass of exactly 1 on the carbon-12 scale (the scale on which the relative masses of atoms are measured). On the carbon-12 scale, a proton has a mass of 1.0073, and a neutron a mass of 1.0087. | |||||||||||||||||
The behaviour of protons, neutrons and electrons in electric fields What happens if a beam of each of these particles is passed between two electrically charged plates - one positive and one negative? Opposites will attract. Protons are positively charged and so would be deflected on a curving path towards the negative plate. Electrons are negatively charged and so would be deflected on a curving path towards the positive plate. Neutrons don't have a charge, and so would continue on in a straight line. Exactly what happens depends on whether the beams of particles enter the electric field with the various particles having the same speeds or the same energies If the particles have the same energy If beams of the three sorts of particles, all with the same energy, are passed between two electrically charged plates:
![]() If the particles have the same speeds If beams of the three sorts of particles, all with the same speed, are passed between two electrically charged plates:
![]() | |||||||||||||||||
Note: This is potentially very confusing! Most chemistry sources that talk about this give either one or the other of these two diagrams without any comment at all - they don't specifically say that they are using constant energy or constant speed beams. But it matters! If this is on your syllabus, it is important that you should know which version your examiners are going to expect, and they probably won't tell you in the syllabus. You should look in detail at past questions, mark schemes and examiner's reports which you can get from your examiners if you are doing a UK-based syllabus. Information about how to do this is on the syllabuses page.If in doubt, I suggest you use the second (constant speed) version. This actually produces more useful information about both masses and charges than the constant energy version. | |||||||||||||||||
The nucleusThe nucleus is at the centre of the atom and contains the protons and neutrons. Protons and neutrons are collectively known as nucleons. Virtually all the mass of the atom is concentrated in the nucleus, because the electrons weigh so little. Working out the numbers of protons and neutrons The atomic number is also given the more descriptive name of proton number. The mass number is also called the nucleon number. This information can be given simply in the form: The atomic number counts the number of protons (9); the mass number counts protons + neutrons (19). If there are 9 protons, there must be 10 neutrons for the total to add up to 19. The atomic number is tied to the position of the element in the Periodic Table and therefore the number of protons defines what sort of element you are talking about. So if an atom has 8 protons (atomic number = 8), it must be oxygen. If an atom has 12 protons (atomic number = 12), it must be magnesium. Similarly, every chlorine atom (atomic number = 17) has 17 protons; every uranium atom (atomic number = 92) has 92 protons. Isotopes The number of neutrons in an atom can vary within small limits. For example, there are three kinds of carbon atom 12C, 13C and 14C. They all have the same number of protons, but the number of neutrons varies.
Isotopes are atoms which have the same atomic number but different mass numbers. They have the same number of protons but different numbers of neutrons. The electronsWorking out the number of electrons Atoms are electrically neutral, and the positiveness of the protons is balanced by the negativeness of the electrons. It follows that in a neutral atom: So, if an oxygen atom (atomic number = 8) has 8 protons, it must also have 8 electrons; if a chlorine atom (atomic number = 17) has 17 protons, it must also have 17 electrons. The arrangement of the electrons The electrons are found at considerable distances from the nucleus in a series of levels called energy levels. Each energy level can only hold a certain number of electrons. The first level (nearest the nucleus) will only hold 2 electrons, the second holds 8, and the third also seems to be full when it has 8 electrons. At GCSE you stop there because the pattern gets more complicated after that. These levels can be thought of as getting progressively further from the nucleus. Electrons will always go into the lowest possible energy level (nearest the nucleus) - provided there is space. To work out the electronic arrangement of an atom
Two important generalisations If you look at the patterns in this table:
In any introductory chemistry course you will have come across the electronic structures of hydrogen and carbon, for example, drawn as: | |||||||||||||||||
Note: There are many places where you could still make use of this model of the atom at A'level. It is, however, a simplification and can be misleading. It gives the impression that the electrons are circling the nucleus in orbits like planets around the sun. As you will find when you look at the A'level view of the atom, it is impossible to know exactly how they are actually moving. | |||||||||||||||||
The circles show energy levels - representing increasing distances from the nucleus. You could straighten the circles out and draw the electronic structure as a simple energy diagram. Carbon, for example, would look like this: | |||||||||||||||||
Fundamental Subatomic Particles
Particle | Symbol | Charge | Mass | |
electron | e- | -1 | 0.0005486 amu | |
proton | p+ | +1 | 1.007276 amu | |
neutron | no | 0 | 1.008665 amu |
- The number of protons in the nucleus of the atom is equal to the atomic number (Z).
- The number of electrons in a neutral atom is equal to the number of protons.
- The mass number of the atom (M) is equal to the sum of the number of protons and neutrons in the nucleus.
- The number of neutrons is equal to the difference between the mass number of the atom (M) and the atomic number (Z).
12C | 13C | 14C | 14N |
12C: 6 electrons, 6 protons, and 6 neutrons
13C: 6 electrons, 6 protons, and 7 neutrons
14C: 6 electrons, 6 protons, and 8 neutrons
Electromagnetic Radiation
Much of what is known about the structure of the electrons in an atom has been obtained by studying the interaction between matter and different forms of electromagnetic radiation. Electromagnetic radiation has some of the properties of both a particle and a wave.
Particles have a definite mass and they occupy space. Waves have no mass and yet they carry energy as they travel through space. In addition to their ability to carry energy, waves have four other characteristic properties: speed, frequency, wavelength, and amplitude. The frequency (v) is the number of waves (or cycles) per unit of time. The frequency of a wave is reported in units of cycles per second (s-1) or hertz (Hz).
The idealized drawing of a wave in the figure below illustrates the definitions of amplitude and wavelength. The wavelength (l) is the smallest distance between repeating points on the wave. The amplitude of the wave is the distance between the highest (or lowest) point on the wave and the center of gravity of the wave.
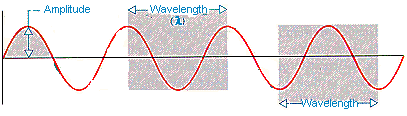
If we measure the frequency (v) of a wave in cycles per second and the wavelength (l) in meters, the product of these two numbers has the units of meters per second. The product of the frequency (v) times the wavelength (l) of a wave is therefore the speed (s) at which the wave travels through space.
vl = s
Electromagnetic Radiation
Much of what is known about the structure of the electrons in an atom has been obtained by studying the interaction between matter and different forms of electromagnetic radiation. Electromagnetic radiation has some of the properties of both a particle and a wave.
Particles have a definite mass and they occupy space. Waves have no mass and yet they carry energy as they travel through space. In addition to their ability to carry energy, waves have four other characteristic properties: speed, frequency, wavelength, and amplitude. The frequency (v) is the number of waves (or cycles) per unit of time. The frequency of a wave is reported in units of cycles per second (s-1) or hertz (Hz).
The idealized drawing of a wave in the figure below illustrates the definitions of amplitude and wavelength. The wavelength (l) is the smallest distance between repeating points on the wave. The amplitude of the wave is the distance between the highest (or lowest) point on the wave and the center of gravity of the wave.
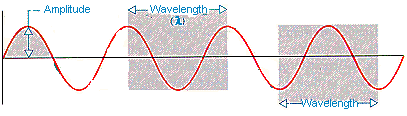
If we measure the frequency (v) of a wave in cycles per second and the wavelength (l) in meters, the product of these two numbers has the units of meters per second. The product of the frequency (v) times the wavelength (l) of a wave is therefore the speed (s) at which the wave travels through space.
vl = s
Electromagnetic Radiation
Much of what is known about the structure of the electrons in an atom has been obtained by studying the interaction between matter and different forms of electromagnetic radiation. Electromagnetic radiation has some of the properties of both a particle and a wave.
Particles have a definite mass and they occupy space. Waves have no mass and yet they carry energy as they travel through space. In addition to their ability to carry energy, waves have four other characteristic properties: speed, frequency, wavelength, and amplitude. The frequency (v) is the number of waves (or cycles) per unit of time. The frequency of a wave is reported in units of cycles per second (s-1) or hertz (Hz).
The idealized drawing of a wave in the figure below illustrates the definitions of amplitude and wavelength. The wavelength (l) is the smallest distance between repeating points on the wave. The amplitude of the wave is the distance between the highest (or lowest) point on the wave and the center of gravity of the wave.
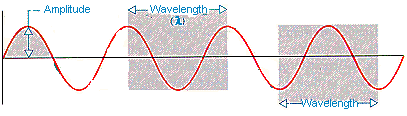
If we measure the frequency (v) of a wave in cycles per second and the wavelength (l) in meters, the product of these two numbers has the units of meters per second. The product of the frequency (v) times the wavelength (l) of a wave is therefore the speed (s) at which the wave travels through space.
vl = s.
Light and Other Forms of Electromagnetic Radiation
Light is a wave with both electric and magnetic components. It is therefore a form of electromagnetic radiation.
Visible light contains the narrow band of frequencies and wavelengths in the portion of the electro-magnetic spectrum that our eyes can detect. It includes radiation with wavelengths between about 400 nm (violet) and 700 nm (red). Because it is a wave, light is bent when it enters a glass prism. When white light is focused on a prism, the light rays of different wavelengths are bent by differing amounts and the light is transformed into a spectrum of colors. Starting from the side of the spectrum where the light is bent by the smallest angle, the colors are red, orange, yellow, green, blue, and violet.
As we can see from the following diagram, the energy carried by light increases as we go from red to blue across the visible spectrum.
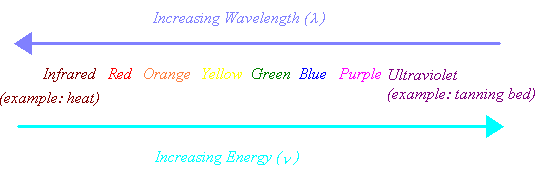
Because the wavelength of electromagnetic radiation can be as long as 40 m or as short as 10-5 nm, the visible spectrum is only a small portion of the total range of electromagnetic radiation.
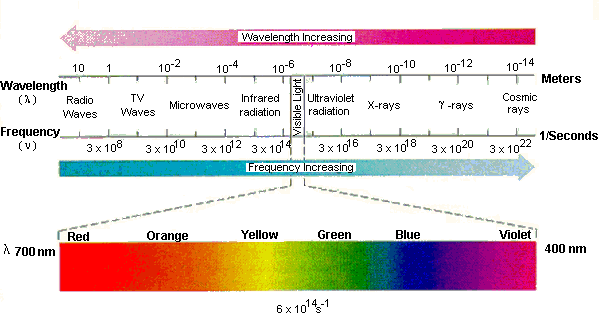
The electromagnetic spectrum includes radio and TV waves, microwaves, infrared, visible light, ultraviolet, x-rays, g-rays, and cosmic rays, as shown in the figure above. These different forms of radiation all travel at the speed of light (c). They differ, however, in their frequencies and wavelengths. The product of the frequency times the wavelength of electromagnetic radiation is always equal to the speed of light.
vl = c
As a result, electromagnetic radiation that has a long wavelength has a low frequency, and radiation with a high frequency has a short wavelength.
ATOM
Matter has mass and takes up space. Atoms are basic building blocks of matter, and cannot be chemically subdivided by ordinary means.
The word atom is derived from the Greek word atom which means indivisible. The Greeks concluded that matter could be broken down into particles to small to be seen. These particles were called atoms
Atoms are composed of three type of particles: protons, neutrons, and electron. Protons and neutrons are responsible for most of the atomic mass e.g in a 150 person 149 lbs, 15 oz are protons and neutrons while only 1 oz. is electrons. The mass of an electron is very small (9.108 X 10-28grams).
Both the protons and neutrons reside in the nucleus. Protons have a postive (+) charge, neutrons have no charge --they are neutral. Electrons reside in orbitals around the nucleus. They have a negative charge (-).
It is the number of protons that determines the atomic number, e.g., H = 1. The number of protons in an element is constant (e.g., H=1, Ur=92) but neutron number may vary, so mass number (protons + neutrons) may vary.
The same element may contain varying numbers of neutrons; these forms of an element are called isotopes. The chemical properties of isotopes are the same, although the physical properties of some isotopes may be different. Some isotopes are radioactive-meaning they "radiate" energy as they decay to a more stable form, perhaps another element half-life: time required for half of the atoms of an element to decay into stable form. Another example is oxygen, with atomic number of 8 can have 8, 9, or 10 neutrons.
What are elements?
All matter is made up of elements which are fundamental substances which cannot be broken down by chemical means. There are 92 elements that occur naturally. The elements hydrogen, carbon, nitrogen and oxygen are the elements that make up most living organisms. Some other elements found in living organisms are: magnesium, calcium, phosphorus, sodium, potassium.
By the late 1800's many elements had already been discovered. The scientist Dmitri Mendeleev, a Russian chemist, proposed an arrangement of know elements based on their atomic mass. The modern arrangement of the elements is known as the Periodic Table of Elements and is arranged according to the atomic number of elements.
What makes each element unique?
Every atom would like to have an electron configuration like a noble gases. In noble gases the outer electron shell is complete. This makes the element chemically inert. Helium is an example of a noble (inert) gas. It is not present in organisms because it is not chemically reactive.
Bohr model shows electrons circling the nucleus at different levels or orbitals much like planets circle the sun. Electrons move from one energy state to another but can only exist aft defineite energy levels. The energy absorbed or released when electrons change states is in the form of electromagnetic radiation.
THE WAVE MODEL AND QUANTUM THEORY
The Bohr model was only able to explain the very simplest atoms, like hydrogen. Today's modern day theory is based on mathematics and the properties of waves. The wave model forms the basis for the Quantum Theory
. This theory gives the probability of locating electrons in a particular location, unlike assuming electrons orbit the nucleus as in the Bohr model.
by:
- losing electrons
- sharing electrons
- gaining electrons.
Atoms that have 1, 2 or 3 electrons in their outer levels will tend to lose them in interactions with atoms that have 5, 6 or 7 electrons in their outer levels. Atoms that have 5, 6 or 7 electrons in their outer levels will tend to gain electrons from atoms with 1, 2 or 3 electrons in their outer levels. Atoms that have 4 electrons in the outer most energy level will tend neither to totally lose nor totally gain electrons during interactions.
This Periodic Table of Elements will show you the electron configuration for any element you click on.




These orbitals were prepared by Dr. Yue-Ling Wong from the University of Florida for more images click here.
MOLECULAR STRUCTURES This page describes how the physical properties of substances having molecular structures varies with the type of intermolecular attractions - hydrogen bonding or van der Waals forces. | ||||||||||||||||||||||
Important! There's not much point in reading this page unless you are reasonably happy about the origin of hydrogen bondingand van der Waals forces. Follow these links first if you aren't sure about these. | ||||||||||||||||||||||
The physical properties of molecular substancesMolecules are made of fixed numbers of atoms joined together by covalent bonds, and can range from the very small (even down to single atoms, as in the noble gases) to the very large (as in polymers, proteins or even DNA). The covalent bonds holding the molecules together are very strong, but these are largely irrelevant to the physical properties of the substance. Physical properties are governed by the intermolecular forces - forces attracting one molecule to its neighbours - van der Waals attractions or hydrogen bonds. Melting and boiling points Molecular substances tend to be gases, liquids or low melting point solids, because the intermolecular forces of attraction are comparatively weak. You don't have to break any covalent bonds in order to melt or boil a molecular substance. | ||||||||||||||||||||||
Note: This is really important! You can make yourself look extremely stupid if you imply in an exam that boiling water, for example, splits it into hydrogen and oxygen by breaking covalent bonds. Exactly the same water molecules are present in ice, water and steam. | ||||||||||||||||||||||
The size of the melting or boiling point will depend on the strength of the intermolecular forces. The presence of hydrogen bonding will lift the melting and boiling points. The larger the molecule the more van der Waals attractions are possible - and those will also need more energy to break. Solubility in water Most molecular substances are insoluble (or only very sparingly soluble) in water. Those which do dissolve often react with the water, or else are capable of forming hydrogen bonds with the water. Why doesn't methane, CH4, dissolve in water? The methane itself isn't the problem. Methane is a gas, and so its molecules are already separate - the water doesn't need to pull them apart from one another. The problem is the hydrogen bonds between the water molecules. If methane were to dissolve, it would have to force its way between water molecules and so break hydrogen bonds. That costs a reasonable amount of energy. The only attractions possible between methane and water molecules are the much weaker van der Waals forces - and not much energy is released when these are set up. It simply isn't energetically profitable for the methane and water to mix. Why does ammonia, NH3, dissolve in water? Ammonia has the ability to form hydrogen bonds. When the hydrogen bonds between water molecules are broken, they can be replaced by equivalent bonds between water and ammonia molecules. Some of the ammonia also reacts with the water to produce ammonium ions and hydroxide ions. Other common substances which are freely soluble in water because they can hydrogen bond with water molecules include ethanol (alcohol) and sucrose (sugar). Solubility in organic solvents Molecular substances are often soluble in organic solvents - which are themselves molecular. Both the solute (the substance which is dissolving) and the solvent are likely to have molecules attracted to each other by van der Waals forces. Although these attractions will be disrupted when they mix, they are replaced by similar ones between the two different sorts of molecules. Electrical conductivity Molecular substances won't conduct electricity. Even in cases where electrons may be delocalised within a particular molecule, there isn't sufficient contact between the molecules to allow the electrons to move through the whole solid or liquid. Some individual examplesIodine, I2 Iodine is a dark grey crystalline solid with a purple vapour. M.Pt: 114°C. B.Pt: 184°C. It is very, very slightly soluble in water, but dissolves freely in organic solvents. Iodine is therefore a low melting point solid. The crystallinity suggests a regular packing of the molecules. The orientation of the iodine molecules within this structure is quite difficult to draw (let alone remember!). If your syllabus and past exam papers suggests that you need to remember it, look carefully at the next sequence of diagrams showing the layers. | ||||||||||||||||||||||
Note: If you are studying a UK-based syllabus and haven't got a copy of yoursyllabus or copies of recent past papers, follow this link to find out how to get them. | ||||||||||||||||||||||
All these diagrams show an "exploded" view of the crystal. The iodine molecules are, of course, touching each other. Measurements of the distances between the centres of the atoms in the crystal show two different values: Ice Ice is a good example of a hydrogen bonded solid. There are lots of different ways that the water molecules can be arranged in ice. This is one of them, but NOT the common one - I can't draw that in any way that makes sense! The one below is known as "cubic ice", or "ice Ic". It is based on the water molecules arranged in a diamond structure. Cubic ice is only stable at temperatures below -80°C. The ice you are familiar with has a different, hexagonal structure. It is called "ice Ih". | ||||||||||||||||||||||
Note: Don't worry about this problem. If asked to draw ice in an exam at this level (16 - 18 year olds), don't try to be too clever. It is probably best not to go beyond the top five molecules in the above diagram. This will show the essential features of the bonding in the structure without getting bogged down in stuff which is far beyond this level.If you are interested in following this up, try a Google search using the search term ice structure hexagonal cubic (or something similar). This will throw up lots of information together with an assortment of fairly dreadful diagrams which I for one don't have the visual imagination to unscramble! | ||||||||||||||||||||||
The unusual density behaviour of water The hydrogen bonding forces a rather open structure on the ice - if you made a model of it, you would find a significant amount of wasted space. When ice melts, the structure breaks down and the molecules tend to fill up this wasted space. This means that the water formed takes up less space than the original ice. Ice is a very unusual solid in this respect - most solids show an increase in volume on melting. When water freezes, the opposite happens - there is an expansion as the hydrogen bonded structure establishes. Most liquids contract on freezing. Remnants of the rigid hydrogen bonded structure are still present in very cold liquid water, and don't finally disappear until 4°C. From 0°C to 4°C, the density of water increases as the molecules free themselves from the open structure and take up less space. After 4°C, the thermal motion of the molecules causes them to move apart and the density falls. That's the normal behaviour with liquids on heating. | ||||||||||||||||||||||
Note: You can find more about water (particularly its abnormally high boiling point) in the page on hydrogen bonding. | ||||||||||||||||||||||
Polymers Bonding in polymers Polymers like poly(ethene) - commonly called polythene - consist of very long molecules. Poly(ethene) molecules are made by joining up lots of ethene molecules into chains of covalently bound carbon atoms with hydrogens attached. There may be short branches along the main chain, also consisting of carbon chains with attached hydrogens. The molecules are attracted to each other in the solid by van der Waals dispersion forces. By controlling the conditions under which ethene is polymerised, it is possible to control the amount of branching to give two distinct types of polythene. High density polythene High density polythene has virtually unbranched chains. The lack of branching allows molecules to lie close together in a regular way which is almost crystalline. Because the molecules lie close together, dispersion forces are more effective, and so the plastic is relatively strong and has a somewhat higher melting point than low density polythene. High density polythene is used for containers for household chemicals like washing-up liquid, for example, or for bowls or buckets. Low density polythene Low density polythene has lots of short branches along the chain. These branches prevent the chains from lying close together in a tidy arrangement. As a result dispersion forces are less and the plastic is weaker and has a lower melting point. Its density is lower, of course, because of the wasted space within the unevenly packed structure. Low density polythene is used for things like plastic bags. The Shape of Molecules
The three dimensional shape or configuration of a molecule is an important characteristic. This shape is dependent on the preferred spatial orientation of covalent bonds to atoms having two or more bonding partners. Three dimensional configurations are best viewed with the aid of models.
Gases, liquids and solids are all made up of microscopic particles, but the behaviors of these particles differ in the three phases. The following figure illustrates the microscopic differences.![]() ![]() hatched bond is directed in back of the plane (away from the viewer), as shown by the bond to substituent D. Some texts and other sources may use a dashed bond in the same manner as we have defined the hatched bond, but this can be confusing because the dashed bond is often used to represent a partial bond (i.e. a covalent bond that is partially formed or partially broken). The following examples make use of this notation, and also illustrate the importance of including non-bonding valence shell electron pairs (colored blue) when viewing such configurations . ![]() ![]() ![]() Methane Ammonia Water Bonding configurations are readily predicted by valence-shell electron-pair repulsion theory, commonly referred to as VSEPR in most introductory chemistry texts. This simple model is based on the fact that electrons repel each other, and that it is reasonable to expect that the bonds and non-bonding valence electron pairs associated with a given atom will prefer to be as far apart as possible. The bonding configurations of carbon are easy to remember, since there are only three categories. Configuration Bonding Partners Bond Angles Example Tetrahedral 4 109.5º ![]() Trigonal 3 120º Linear 2 180º In the three examples shown above, the central atom (carbon) does not have any non-bonding valence electrons; consequently the configuration may be estimated from the number of bonding partners alone. For molecules of water and ammonia, however, the non-bonding electrons must be included in the calculation. In each case there are four regions of electron density associated with the valence shell so that a tetrahedral bond angle is expected. The measured bond angles of these compounds (H2O 104.5º & NH3 107.3º) show that they are closer to being tetrahedral than trigonal or linear. Of course, it is the configuration of atoms (not electrons) that defines the the shape of a molecule, and in this sense ammonia is said to be pyramidal (not tetrahedral). The compound boron trifluoride, BF3, does not have non-bonding valence electrons and the configuration of its atoms is trigonal. Nice treatments of VSEPR theory have been provided by Oxford and Purdue . Click on the university name to visit their site. The best way to study the three-dimensional shapes of molecules is by using molecular models. Many kinds of model kits are available to students and professional chemists. Some of the useful features of physical models can be approximated by the model viewing applet Jmol. This powerful visualization tool allows the user to move a molecular stucture in any way desired. Atom distances and angles are easily determined. To measure a distance, double-click on two atoms. To measure a bond angle, do a double-click, single-click, double-click on three atoms Isomers Structural Formulas It is necessary to draw structural formulas for organic compounds because in most cases a molecular formula does not uniquely represent a single compound. Different compounds having the same molecular formula are called isomers, and the prevalence of organic isomers reflects the extraordinary versatility of carbon in forming strong bonds to itself and to other elements. When the group of atoms that make up the molecules of different isomers are bonded together in fundamentally different ways, we refer to such compounds as constitutional isomers. There are seven constitutional isomers of C4H10O, and structural formulas for these are drawn in the following table. These formulas represent all known and possible C4H10O compounds, and display a common structural feature. There are no double or triple bonds and no rings in any of these structures.. Note that each of the carbon atoms is bonded to four other atoms, and is saturated with bonding partners. Structural Formulas for C4H10O Isomers Kekulé Formula Condensed Formula Shorthand Formula ![]() Simplification of structural formulas may be achieved without any loss of the information they convey. In condensed structural formulas the bonds to each carbon are omitted, but each distinct structural unit (group) is written with subscript numbers designating multiple substituents, including the hydrogens. Shorthand (line) formulas omit the symbols for carbon and hydrogen entirely. Each straight line segment represents a bond, the ends and intersections of the lines are carbon atoms, and the correct number of hydrogens is calculated from the tetravalency of carbon. Non-bonding valence shell electrons are omitted in these formulas. Developing the ability to visualize a three-dimensional structure from two-dimensional formulas requires practice, and in most cases the aid of molecular models. As noted earlier, many kinds of model kits are available to students and professional chemists, and the beginning student is encouraged to obtain one. Analysis of Molecular Formulas Although structural formulas are essential to the unique description of organic compounds, it is interesting and instructive to evaluate the information that may be obtained from a molecular formula alone. Three useful rules may be listed: The number of hydrogen atoms that can be bonded to a given number of carbon atoms is limited by the valence of carbon. For compounds of carbon and hydrogen (hydrocarbons) the maximum number of hydrogen atoms that can be bonded to n carbons is 2n + 2 (n is an integer). In the case of methane, CH4, n=1 & 2n + 2 = 4. The origin of this formula is evident by considering a hydrocarbon made up of a chain of carbon atoms. Here the middle carbons will each have two hydrogens and the two end carbons have three hydrogens each. Thus, a six-carbon chain (n = 6) may be written H-(CH2)6-H, and the total hydrogen count is (2 x 6) + 2 = 14. The presence of oxygen (valence = 2) does not change this relationship, so the previously described C4H10O isomers follow the rule, n=4 &2n + 2 = 10. Halogen atoms (valence = 1) should be counted equivalent to hydrogen, as illustrated by C3H5Cl3, n = 3 & 2n + 2 = 8 = (5 + 3). If nitrogen is present, each nitrogen atom (valence = 3) increases the maximum number of hydrogens by one. Some Plausible Molecular Formulas C7H16O3, C9H18, C15H28O3, C6H16N2 Some Impossible Molecular Formulas C8H20O6, C23H50, C5H10Cl4, C4H12NO For stable organic compounds the total number of odd-valenced atoms is even. Thus, when even-valenced atoms such as carbon and oxygen are bonded together in any number and in any manner, the number of remaining unoccupied bonding sites must be even. If these sites are occupied by univalent atoms such as H, F, Cl, etc. their total number will necessarily be even. Nitrogen is also an odd-valenced atom (3), and if it occupies a bonding site on carbon it adds two additional bonding sites, thus maintaining the even/odd parity. Some Plausible Molecular Formulas C4H4Cl2, C5H9OBr, C5H11NO2, C12H18N2FCl Some Impossible Molecular Formulas C5H9O2, C4H5ClBr, C6H11N2O, C10H18NCl2 The number of hydrogen atoms in stable compounds of carbon, hydrogen & oxygen reflects the number of double bonds and rings in their structural formulas. Consider a hydrocarbon with a molecular structure consisting of a simple chain of four carbon atoms, CH3CH2CH2CH3. The molecular formula is C4H10 (the maximum number of bonded hydrogens by the 2n + 2 rule). If the four carbon atoms form a ring, two hydrogens must be lost. Similarly, the introduction of a double bond entails the loss of two hydrogens, and a triple bond the loss of four hydrogens. ![]() From the above discussion and examples it should be clear that the molecular formula of a hydrocarbon (CnHm) provides information about the number of rings and/or double bonds that must be present in its structural formula. By rule #2 m must be an even number, so if m < (2n + 2) the difference is also an even number that reflects any rings and double bonds. A triple bond is counted as two double bonds. ![]() The presence of one or more nitrogen atoms or halogen substituents requires a modified analysis. Resonance Kekulé structural formulas are essential tools for understanding organic chemistry. However, the structures of some compounds and ions cannot be represented by a single formula. For example, sulfur dioxide (SO2) and nitric acid (HNO3) may each be described by two equivalent formulas (equations 1 & 2). For clarity the two ambiguous bonds to oxygen are given different colors in these formulas. 1) sulfur dioxide ![]() 2) nitric acid If only one formula for sulfur dioxide was correct and accurate, then the double bond to oxygen would be shorter and stronger than the single bond. Since experimental evidence indicates that this molecule is bent (bond angle 120º) and has equal length sulfur : oxygen bonds (1.432 Å), a single formula is inadequate, and the actual structure resembles an average of the two formulas. This averaging of electron distribution over two or more hypothetical contributing structures (canonical forms) to produce a hybrid electronic structure is called resonance. Likewise, the structure of nitric acid is best described as aresonance hybrid of two structures, the double headed arrow being the unique symbol for resonance. The above examples represent one extreme in the application of resonance. Here, two structurally and energetically equivalent electronic structures for a stable compound can be written, but no single structure provides an accurate or even an adequate representation of the true molecule. In cases such as these, the electron delocalization described by resonance enhances the stability of the molecules, and compounds or ions composed of such molecules often show exceptional stability. 3) formaldehyde ![]() The electronic structures of most covalent compounds do not suffer the inadequacy noted above. Thus, completely satisfactory Kekulé formulas may be drawn for water (H2O), methane (CH4) and acetylene C2H2). Nevertheless, the principles of resonance are very useful in rationalizing the chemical behavior of many such compounds. For example, the carbonyl group of formaldehyde (the carbon-oxygen double bond) reacts readily to give addition products. The course of these reactions can be explained by a small contribution of a dipolar resonance contributor, as shown in equation 3. Here, the first contributor (on the left) is clearly the best representation of this molecular unit, since there is no charge separation and both the carbon and oxygen atoms have achieved valence shell neon-like configurations by covalent electron sharing. If the double bond is broken heterolytically, formal charge pairs result, as shown in the other two structures. The preferred charge distribution will have the positive charge on the less electronegative atom (carbon) and the negative charge on the more electronegative atom (oxygen). Therefore the middle formula represents a more reasonable and stable structure than the one on the right. The application of resonance to this case requires a weighted averaging of these canonical structures. The double bonded structure is regarded as the major contributor, the middle structure a minor contributor and the right hand structure a non-contributor. Since the middle, charge-separated contributor has an electron deficient carbon atom, this explains the tendency of electron donors (nucleophiles) to bond at this site. The basic principles of the resonance method may now be summarized. For a given compound, a set of Lewis / Kekulé structures are written, keeping the relative positions of all the component atoms the same. These are the canonical forms to be considered, and all must have the same number of paired and unpaired electrons. The following factors are important in evaluating the contribution each of these canonical structures makes to the actual molecule. The number of covalent bonds in a structure. (The greater the bonding, the more important and stable the contributing structure.) Formal charge separation. (Other factors aside, charge separation decreases the stability and importance of the contributing structure.) Electronegativity of charge bearing atoms and charge density. (High charge density is destabilizing. Positive charge is best accommodated on atoms of low electronegativity, and negative charge on high electronegative atoms.) The stability of a resonance hybrid is always greater than the stability of any canonical contributor. Consequently, if one canonical form has a much greater stability than all others, the hybrid will closely resemble it electronically and energetically. This is the case for the carbonyl group (eq.3). The left hand C=O structure has much greater total bonding than either charge-separated structure, so it describes this functional group rather well. On the other hand, if two or more canonical forms have identical low energy structures, the resonance hybrid will have exceptional stabilization and unique properties. This is the case for sulfur dioxide (eq.1) and nitric acid (eq.2). 4) carbon monoxide ![]() 5) azide anion To illustrate these principles we shall consider carbon monoxide (eq.4) and azide anion (eq.5). In each case the most stable canonical form is on the left. For carbon monoxide, the additional bonding is more important than charge separation. Furthermore, the double bonded structure has an electron deficient carbon atom (valence shell sextet). A similar destabilizing factor is present in the two azide canonical forms on the top row of the bracket (three bonds vs. four bonds in the left most structure). The bottom row pair of structures have four bonds, but are destabilized by the high charge density on a single nitrogen atom. Atomic and Molecular Orbitals A more detailed model of covalent bonding requires a consideration of valence shell atomic orbitals. For second period elements such as carbon, nitrogen and oxygen, these orbitals have been designated 2s, 2px, 2py & 2pz. The spatial distribution of electrons occupying each of these orbitals is shown in the diagram below. Very nice displays of orbitals may be found at the following sites: J. Gutow, Univ. Wisconsin Oshkosh R. Spinney, Ohio State M. Winter, Sheffield University ![]() The valence shell electron configuration of carbon is 2s2, 2px1, 2py1 & 2pz0. If this were the configuration used in covalent bonding, carbon would only be able to form two bonds. In this case, the valence shell would have six electrons- two shy of an octet. However, the tetrahedral structures of methane and carbon tetrachloride demonstrate that carbon can form four equivalent bonds, leading to the desired octet. In order to explain this covalent bonding, Linus Pauling proposed an orbital hybridization model in which all the valence shell electrons of carbon are reorganized. Hybrid Orbitals In order to explain the structure of methane (CH4), the 2s and three 2p orbitals are converted to four equivalent hybrid atomic orbitals, each having 25% s and 75% p character, and designated sp3. These hybrid orbitals have a specific orientation, and the four are naturally oriented in a tetrahedral fashion. Thus, the four covalent bonds of methane consist of shared electron pairs with four hydrogen atoms in a tetrahedral configuration, as predicted by VSEPR theory. ![]() Molecular Orbitals Just as the valence electrons of atoms occupy atomic orbitals (AO), the shared electron pairs of covalently bonded atoms may be thought of as occupying molecular orbitals (MO). It is convenient to approximate molecular orbitals by combining or mixing two or more atomic orbitals. In general, this mixing of n atomic orbitals always generates n molecular orbitals. The hydrogen molecule provides a simple example of MO formation. In the following diagram, two 1s atomic orbitals combine to give a sigma (σ) bonding (low energy) molecular orbital and a second higher energy MO referred to as an antibonding orbital. The bonding MO is occupied by two electrons of opposite spin, the result being a covalent bond. ![]() The notation used for molecular orbitals parallels that used for atomic orbitals. Thus, s-orbitals have a spherical symmetry surrounding a single nucleus, whereas σ-orbitals have a cylindrical symmetry and encompass two (or more) nuclei. In the case of bonds between second period elements, p-orbitals or hybrid atomic orbitals having p-orbital character are used to form molecular orbitals. For example, the sigma molecular orbital that serves to bond two fluorine atoms together is generated by the overlap of p-orbitals (part A below), and two sp3 hybrid orbitals of carbon may combine to give a similar sigma orbital. When these bonding orbitals are occupied by a pair of electrons, a covalent bond, the sigma bond results. Although we have ignored the remaining p-orbitals, their inclusion in a molecular orbital treatment does not lead to any additional bonding, as may be shown by activating the fluorine correlation diagram below. ![]() Another type of MO (the π orbital) may be formed from two p-orbitals by a lateral overlap, as shown in part A of the following diagram. Since bonds consisting of occupied π-orbitals (pi-bonds) are weaker than sigma bonds, pi-bonding between two atoms occurs only when a sigma bond has already been established. Thus, pi-bonding is generally found only as a component of double and triple covalent bonds. Since carbon atoms involved in double bonds have only three bonding partners, they require only three hybrid orbitals to contribute to three sigma bonds. A mixing of the 2s-orbital with two of the 2p orbitals gives three sp2 hybrid orbitals, leaving one of the p-orbitals unused. Two sp2 hybridized carbon atoms are then joined together by sigma and pi-bonds (a double bond), as shown in part B. ![]() The manner in which atomic orbitals overlap to form molecular orbitals is actually more complex than the localized examples given above. These are useful models for explaining the structure and reactivity of many organic compounds, but modern molecular orbital theory involves the creation of an orbital correlation diagram. Two examples of such diagrams for the simple diatomic elements F2 and N2 will be drawn above when the appropriate button is clicked. The 1s and 2s atomic orbitals do not provide any overall bonding, since orbital overlap is minimal, and the resulting sigma bonding and antibonding components would cancel. In both these cases three 2p atomic orbitals combine to form a sigma and two pi-molecular orbitals, each as a bonding and antibonding pair. The overall bonding order depends on the number of antibonding orbitals that are occupied. The subtle change in the energy of the σ2p bonding orbital, relative to the two degenerate π-bonding orbitals, is due to s-p hybridization that is unimportant to the present discussion. The p-orbitals in these model are represented by red and blue colored spheres or ellipses, which represent different phases, defined by the mathematical wave equations for such orbitals. Finally, in the case of carbon atoms with only two bonding partners only two hybrid orbitals are needed for the sigma bonds, and these sp hybrid orbitals are directed 180º from each other. Two p-orbitals remain unused on each sp hybridized atom, and these overlap to give two pi-bonds following the formation of a sigma bond (a triple bond), as shown below. ![]()
Liquids and solids are often referred to as condensed phases because the particles are very close together.
The following table summarizes properties of gases, liquids, and solids and identifies the microscopic behavior responsible for each property.
What is a solution? |
We make the custom synthesis process more efficient and cost effective while maintaining the highest standards of quality and reliability. 1-pentyl-3-methylimidazolium trifluoroacetate
ReplyDelete